
Single particle cryo-electron microscopy (cryo-EM) offers a powerful way to study biological molecules, but its success depends heavily on the preparation of the cryo-grid. This intricate process begins with 3 µl of a biological molecule suspended in water or buffer at room temperature or 4°C, which is then carefully deposited onto a grid roughly 3 mm in diameter. After application, the grid and sample undergo a rapid plunge into liquid ethane cooled by liquid nitrogen, freezing them instantly at a temperature of -180°C (93K) (this is what gives it the name "cryo"-EM). If a sample looks promising in a test tube and during negative stain but appears suboptimal on a cryo-grid, it’s usually due to the environmental interfaces or to the physical mechanisms involved in cryo-grid preparation.
At the heart of cryo-grid preparation are three critical physical changes:
- The proximity of the sample to the grid
- A reduction in water volume
- An extreme temperature drop
In the following sections, we'll explore these changes in more detail and discuss how they influence the sample and, ultimately, the success of the cryo-EM experiment. However, it is important to emphasize that thorough biochemical characterization of the sample before cryo-grid preparation is crucial, as it ensures that any issues with sample appearance on the grid can be attributed to the preparation process, rather than underlying problems with the sample itself.
Understanding the Grid and Water Changes During Cryo-Grid Production
The Anatomy of a Grid
The grid is the stage on which your sample will be placed, and its material composition plays a crucial role in how well the sample behaves during preparation. Most grids are made from carbon, gold, or copper, and they consist of two main layers. The first is the gridbar layer, a mesh of bars arranged in a perpendicular pattern, which creates a framework of open squares, as shown in Figure 1 (below). The second layer is a much thinner layer of foil, typically made of carbon, gold, or occasionally other materials. This delicate foil is perforated with tiny holes and is usually hydrophobic, but can be treated to increase its hydrophilicity to ensure the sample spreads evenly across the surface. This treatment involves exposing the grid to a plasma environment, where charged molecules interact with the foil’s surface to alter its properties.
The final, optional grid component is an additional continuous layer on top of the holey foil. This film, made from materials like amorphous carbon or graphene, can make a significant difference in how well the sample behaves and will be discussed further in a later section. Changing the material of a grid, the spacing and size of the holes in the holey foil, or adding a continuous support film on top of the holey foil can sometimes be the key to successful sample preparation and imaging in cryo-EM.
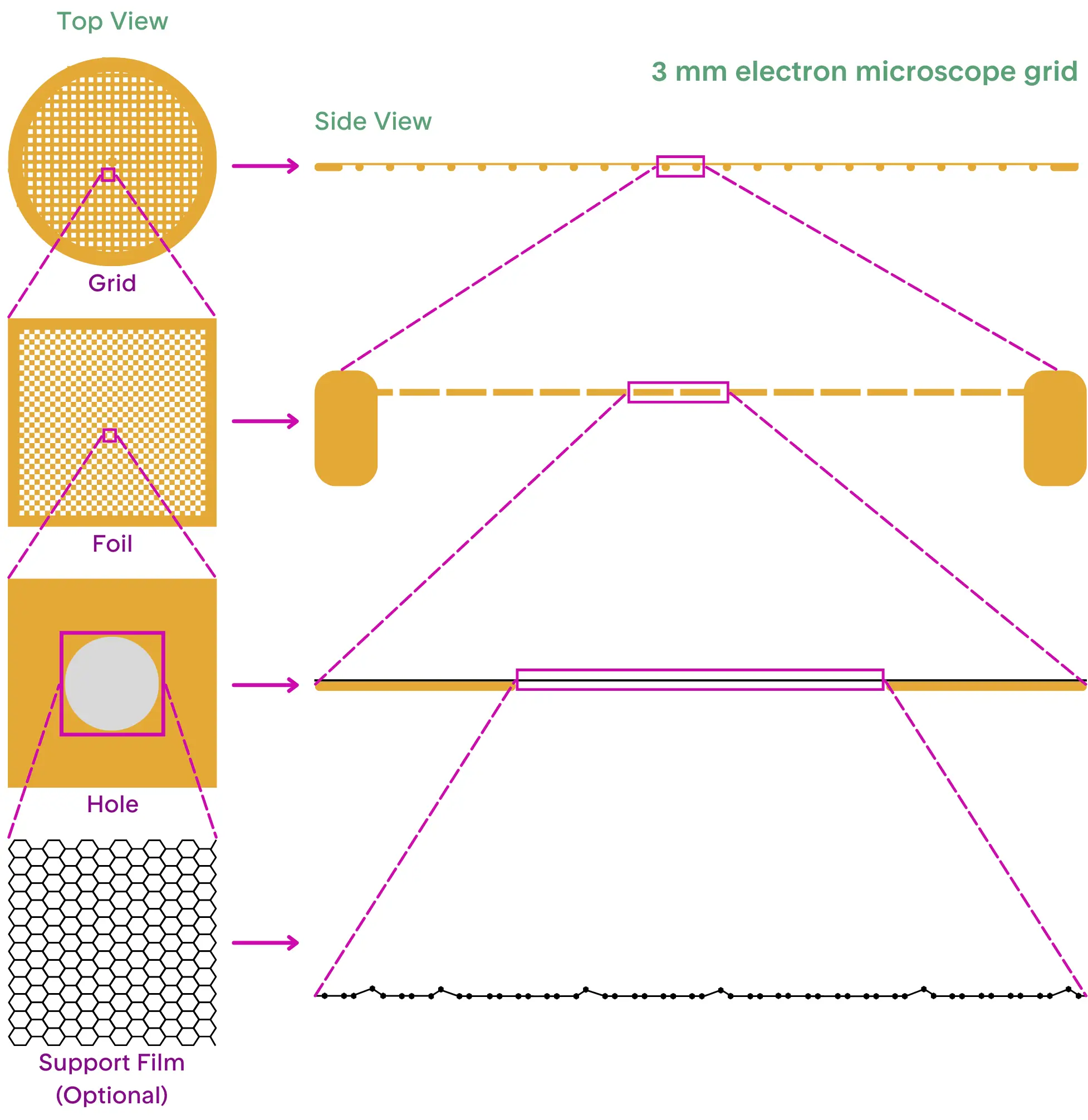
The Behavior of Water on the Grid
The water in which the sample is suspended undergoes a dramatic transformation during cryo-grid preparation. In pure water (or bulk water), the molecules are highly ordered in a tetrahedral structure, yet they remain dynamic. When water molecules come into contact with a non-water component, their order and dynamics change, resulting in a structurally distinct layer of water surrounding the non-water surface[1, 2]. Once a sample is placed on the grid, the water becomes confined to a thin layer (about 50-100 nm thick) within the grid's holes. The interaction between water and air or water and grid material in this thin layer of sample will significantly alter the behavior of the water molecules[3, 4, 5, 6]. These changes at the water-air interface play a crucial role in determining the outcome of cryo-EM sample preparation[7, 8].
The Freeze
The key to capturing high-quality cryo-EM images is the preservation of biological molecules in a state known as vitreous ice—a form of water that freezes without forming crystals. This is achieved by plunging the grid from room temperature to -180°C within seconds[9]. This rapid cooling prevents the formation of crystalline ice, allowing the water molecules to retain their flexible tetrahedral network. The biological molecules suspended in the water are thus trapped in their native, unaltered state, which is essential for accurate imaging[10, 11].
The Complex Interplay Between the Grid, Water, and Sample
Protein Behavior at the Air-Water Interface (AWI)
The protein of interest is the central player in cryo-grid preparation, as its interactions with water and the grid materials can significantly impact the outcome of the experiment. One of the most important factors in this process is the protein's hydration shell—the thin layer of water molecules that closely interacts with the protein's surface. This shell is vital for maintaining the protein's stability and ensuring it remains properly folded in its functional conformation.
During cryo-grid preparation, as the sample is deposited onto the grid, excess water is blotted away, which changes the ratio of protein to water. This process exposes the protein to a larger air-water interface (AWI), where the delicate hydration shell may be disrupted. A layer of denatured or partially denatured protein, up to ~14 nm thick, is likely immediately created after blotting, which is believed to occur because the rate of diffusion of a sample to the interface (less than a second to maybe nanoseconds) is faster than the speed of freezing ([12, 13, 14, 15]. The creation of this protein layer is still not fully understood and may vary between different samples.
Understanding protein behavior at the air-water interface (AWI) is essential for optimal cryo-grid preparation. The AWI plays a pivotal role in determining sample stability during grid production, often explaining the disparity between a sample's appearance before cryo-grid preparation and its quality during imaging. When proteins interact with this interface, they can undergo denaturation or aggregation, which negatively impacts the final image quality. The AWI is widely recognized as a major contributor to these issues, with its effects clearly demonstrated by tomographic studies of grids containing purified proteins with some of the observations illustrated in Figure 2[8]. However, we still have no universal explanation for why these effects happen consistently across different samples.

Improving Sample Behavior at the AWI
Fortunately, there are ways to mitigate issues at the AWI. One common strategy is to add detergents to the sample. Detergents interact with the AWI by inserting their hydrophilic heads into the water and their hydrophobic tails into the air, disrupting the protein’s interaction with the interface and potentially preventing denaturation. Surfactants like amphipols and chaperone proteins (such as LEA proteins) can also interact with proteins and their hydration shell to minimize damage during the grid preparation process[16, 17]. Detergents gather around proteins to stabilize hydrophobic patches, while LEA chaperones’ naturally bind to the AWI to protect proteins[19]. Another approach involves using different buffers or salts to stabilize the sample in solution before applying it to the grid.
Alternatively, advances in grid vitrification systems aim to freeze the grids faster, decreasing the diffusion time from seconds to milliseconds and reducing the time proteins spend exposed to the AWI[20, 21]. However, many of these systems are still under development and may not be universally effective.
The Role of Grid Materials
The material of the grid itself can also have a significant impact on sample behavior. For instance, some samples may behave differently on grids made of gold versus carbon. As there is still a lot to learn about how different grid materials interact with specific samples, it’s common practice to test multiple types of grids as a first step to find the one that works best for a given sample.
Continuous Support Films: A Promising Solution
One of the most promising innovations in cryo-EM sample preparation is the use of continuous support films. These films help stabilize proteins by providing a more consistent interface for them to adsorb onto, eliminating one of the two air-water interfaces (Figure 3). Continuous support films are most commonly made from carbon-based materials, although some have also been made using 2D crystals of streptavidin protein which are difficult to prepare and may take years to optimize[18]. The carbon support films can range in thickness from 2 to 4 nm of multiple, amorphous, non-ordered layers to a single atomic layer of graphene.
Amorphous carbon is a layer created by sputtering carbon onto mica and transferring it onto a grid's holey foil. Grids with a continuous amorphous carbon layer are widely available and provide consistent coverage, making them ideal for data collection. However, while the film ensures even coverage, it also introduces substantial background noise that can drown out the signal from the protein of interest.
Graphene is a single layer of carbon atoms that is thin, strong, chemically flat, and has high thermal and electrical conductivity, offering minimal background noise while stabilizing proteins. However, in its pristine form, graphene is inert and does not interact easily with proteins. To make it suitable for biological applications, graphene can be modified through gentle treatments like shorter plasma exposure or UV/Ozone, though harsher methods may damage it[23, 24]. More tailored plasma chemical modifications can introduce functional groups such as nitrile, carboxyl, or thiol, but specialized equipment for these treatments is often expensive and limits their widespread use[22]. Other methods include wet chemical functionalization or adding molecules to the graphene surface[25, 26].
The main challenge in using graphene grids is producing a continuous single layer of graphene, although methods using graphene oxide flakes are improving. Oxidized graphene flakes can be suspended in liquid and allowed to assemble as a continuous layer on a holey foil[27]. The oxidation turns the graphene into a surface that can interact with proteins and be further modified[28]. While the interface created is nearly ideal, issues can arise if the flakes stack into multiple layers or fail to cover the entire grid. Such inconsistencies reduce the effective imaging area, limiting the grid’s suitability for data collection.

Conclusion
Cryo-grid preparation is a complex process with many variables that can impact the outcome, which helps us understand why even subtle changes to sample preparation can lead to dramatic differences in the results. If a sample appears promising in a test tube and under negative staining but looks suboptimal on a cryo-grid, it is often due to the intricate interactions between the sample, grid materials, water, and the air-water interface during vitrification. Despite efforts to characterize the physical changes that occur during freezing, many aspects of this process remain poorly understood, making it difficult to predict the results. Therefore, to achieve the best results, it’s essential that each sample is well-characterized and stable in solution, and that extensive cryo-grid screening and optimization are performed for new samples. Future advancements in cryo-grid production will likely focus on refining grid materials, support films, and sample treatments to better control conditions and maximize the potential of cryo-EM. While the process may seem unpredictable at times, it’s clear that with careful screening, optimization, and the right tools, successful cryo-EM imaging can be achieved.
Contact us to discuss your project today.
Citations & References
1. Torres, J., Buck, Z. N., Kaiser, H., He, X., White, T., Tyagi, M., Winholtz, R. A., Hansen, F. Y., Herwig, K. W., & Taub, H. (2019). A neutron scattering and electron microscopy study of the structure, wetting, and freezing behavior of water near hydrophilic CuO-nanostructured surfaces. Journal of Applied Physics, 125(2), 025302. https://doi.org/10.1063/1.5060976
2. Akaishi, A., Yonemaru, T., & Nakamura, J. (2017). Formation of Water Layers on Graphene Surfaces. ACS Omega, 2(5), 2184–2190. https://doi.org/10.1021/acsomega.7b00365
3. Martins-Costa, M. T. C., & Ruiz-López, M. F. (2023). Electrostatics and Chemical Reactivity at the Air–Water Interface. Journal of the American Chemical Society, 145(2), 1400–1406. https://doi.org/10.1021/jacs.2c12089
4. Goswami, M., Kumar, N., Li, Y., Rios, O., Akamo, D. O., Hirschey, J., LaClair, T. J., & Gluesenkamp, K. R. (2021). Comparison of water nanodroplet properties on different graphite-based substrates. AIP Advances, 11(3), 035009. https://doi.org/10.1063/5.0042414
5. Bhowmik, D., Ganesh, P., Sumpter, B. G., & Goswami, M. (2018). Dynamical disparity between hydration shell water and RNA in a hydrated RNA system. Physical Review E, 98(6), 62407. https://doi.org/10.1103/PhysRevE.98.062407
6. Buchsteiner, Alexandra et al. “Water dynamics in graphite oxide investigated with neutron scattering.” The journal of physical chemistry. B vol. 110,45 (2006): 22328-38. doi:10.1021/jp0641132
7. Li, Bufan et al. “Effect of charge on protein preferred orientation at the air-water interface in cryo-electron microscopy.” Journal of structural biology vol. 213,4 (2021): 107783. doi:10.1016/j.jsb.2021.107783
8. Noble, A.J., Wei, H., Dandey, V.P. et al. Reducing effects of particle adsorption to the air–water interface in cryo-EM. Nat Methods 15, 793–795 (2018). https://doi.org/10.1038/s41592-018-0139-3
9. Dubochet, J., Adrian, M., Chang, J.-J., Homo, J.-C., Lepault, J., McDowall, A. W., & Schultz, P. (1988). Cryo-electron microscopy of vitrified specimens. Quarterly Reviews of Biophysics, 21(2), 129–228. https://doi.org/DOI: 10.1017/S0033583500004297
10. Parkhurst, J. M., Cavalleri, A., Dumoux, M., Basham, M., Clare, D., Siebert, C. A., Evans, G., Naismith, J. H., Kirkland, A., & Essex, J. W. (2024). Computational models of amorphous ice for accurate simulation of cryo-EM images of biological samples. Ultramicroscopy, 256, 113882. https://doi.org/https://doi.org/10.1016/j.ultramic.2023.113882
11. Eltareb, A., Lopez, G. E., & Giovambattista, N. (2021). The role of high-density and low-density amorphous ice on biomolecules at cryogenic temperatures: a case study with polyalanine. Physical Chemistry Chemical Physics, 23(35), 19402–19414. https://doi.org/10.1039/D1CP02734D
12. Yano, Y. F., Arakawa, E., Voegeli, W., & Matsushita, T. (2013). Real-time investigation of protein unfolding at an air-water interface at the 1 s time scale. Journal of Synchrotron Radiation, 20(6), 980–983. https://doi.org/10.1107/S0909049513023741
13. Raffaini, G., & Ganazzoli, F. (2010). Protein Adsorption on a Hydrophobic Surface: A Molecular Dynamics Study of Lysozyme on Graphite. Langmuir, 26(8), 5679–5689. https://doi.org/10.1021/la903769c
14. Kudryashova, E.V., Visser, A.J.W.G. and De Jongh, H.H.J. (2005), Reversible self-association of ovalbumin at air–water interfaces and the consequences for the exerted surface pressure. Protein Science, 14: 483-493. https://doi.org/10.1110/ps.04771605
15. James, L. K., & Augenstein, L. G. (1966). Adsorption of Enzymes at Interfaces: Film Formation and the Effect on Activity. In Advances in Enzymology and Related Areas of Molecular Biology (pp. 1–40). https://doi.org/https://doi.org/10.1002/9780470122730.ch1
16. Abe, K. M., & Lim, C. J. (2024). Small LEA proteins as an effective air-water interface protectant for fragile samples during cryo-EM grid plunge freezing. Nature communications, 15(1), 7705. https://doi.org/10.1038/s41467-024-52091-1
17. Michon, Baptiste et al. “Role of surfactants in electron cryo-microscopy film preparation.” Biophysical journal vol. 122,10 (2023): 1846-1857. doi:10.1016/j.bpj.2023.04.016
18. Han, B.-G., Watson, Z., Kang, H., Pulk, A., Downing, K. H., Cate, J., & Glaeser, R. M. (2016). Long shelf-life streptavidin support-films suitable for electron microscopy of biological macromolecules. Journal of Structural Biology, 195(2), 238–244. https://doi.org/https://doi.org/10.1016/j.jsb.2016.06.009
19. Rendón-Luna, D. F., Arroyo-Mosso, I. A., de Luna-Valenciano, H., Campos, F., Segovia, L., Saab-Rincón, G., Cuevas-Velazquez, C. L., Reyes, J. L., & Covarrubias, A. A. (2024). Alternative conformations of a group 4 Late Embryogenesis Abundant protein associated to its in vitro protective activity. Scientific Reports, 14(1), 2770. https://doi.org/10.1038/s41598-024-53295-7
20. Razinkov, I., Dandey, V. P., Wei, H., Zhang, Z., Melnekoff, D., Rice, W. J., Wigge, C., Potter, C. S., & Carragher, B. (2016). A new method for vitrifying samples for cryoEM. Journal of Structural Biology, 195(2), 190–198. https://doi.org/https://doi.org/10.1016/j.jsb.2016.06.001
21. Jain, T., Sheehan, P., Crum, J., Carragher, B., & Potter, C. S. (2012). Spotiton: A prototype for an integrated inkjet dispense and vitrification system for cryo-TEM. Journal of Structural Biology, 179(1), 68–75. https://doi.org/https://doi.org/10.1016/j.jsb.2012.04.020
22. Naydenova, K., Peet, M. J., & Russo, C. J. (2019). Multifunctional graphene supports for electron cryomicroscopy. Proceedings of the National Academy of Sciences, 116(24), 11718–11724. https://doi.org/10.1073/pnas.1904766116
23. Sharma, K., López-Sánchez, U., Nury, H., Schoehn, G., Darnault, C., Breyton, C., Petit-Etienne, C., Vergnaud, C., Ling, W. L., Cunge, G., & Okuno, H. (2024). Precision defect integrated graphene as reliable support membrane for high-resolution cryo-transmission electron microscopy. Carbon, 230. https://doi.org/10.1016/j.carbon.2024.119625
24. Han, Y., Fan, X., Wang, H., Zhao, F., Tully, C. G., Kong, J., Yao, N., & Yan, N. (2020). High-yield monolayer graphene grids for near-atomic resolution cryoelectron microscopy. Proceedings of the National Academy of Sciences, 117(2), 1009–1014. https://doi.org/10.1073/pnas.1919114117
25. Lu, Y., Liu, N., Liu, Y., Zheng, L., Yang, J., Wang, J., Jia, X., Zi, Q., Peng, H., Rao, Y., & Wang, H. W. (2022). Functionalized graphene grids with various charges for single-particle cryo-EM. Nature Communications, 13(1). https://doi.org/10.1038/s41467-022-34579-w
26. Zheng, L., Xu, J., Wang, W., Gao, X., Zhao, C., Guo, W., Sun, L., Cheng, H., Meng, F., Chen, B., Sun, W., Jia, X., Zhou, X., Wu, K., Liu, Z., Ding, F., Liu, N., Wang, H. W., & Peng, H. (2024). Self-assembled superstructure alleviates air-water interface effect in cryo-EM. Nature Communications, 15(1). https://doi.org/10.1038/s41467-024-51696-w
27. Palovcak, E., Wang, F., Zheng, S. Q., Yu, Z., Li, S., Betegon, M., Bulkley, D., Agard, D. A., & Cheng, Y. (2018). A simple and robust procedure for preparing graphene-oxide cryo-EM grids. Journal of structural biology, 204(1), 80–84. https://doi.org/10.1016/j.jsb.2018.07.007
28. Chio, U. S., Palovcak, E., Smith, A. A. A., Autzen, H., Muñoz, E. N., Yu, Z., Wang, F., Agard, D. A., Armache, J. P., Narlikar, G. J., & Cheng, Y. (2024). Functionalized graphene-oxide grids enable high-resolution cryo-EM structures of the SNF2h-nucleosome complex without crosslinking. Nature Communications, 15(1). https://doi.org/10.1038/s41467-024-46178-y

Welcome to Finsweet's accessible modal component for Webflow Libraries. This modal uses Webflow Interactions to open and close. It is accessible through custom attributes and custom JavaScript added in the embed block of the component. If you're interested in how this is built, check out the Attributes documentation page for this modal component.
Infographic Available for Download
Learn more about materials and interfaces, and their impacts on cryo electron microscopy sample preparation.
